What is the Standard Model?
The Standard Model is our best theory for how the universe operates, but there are some missing pieces that physicists are struggling to find.
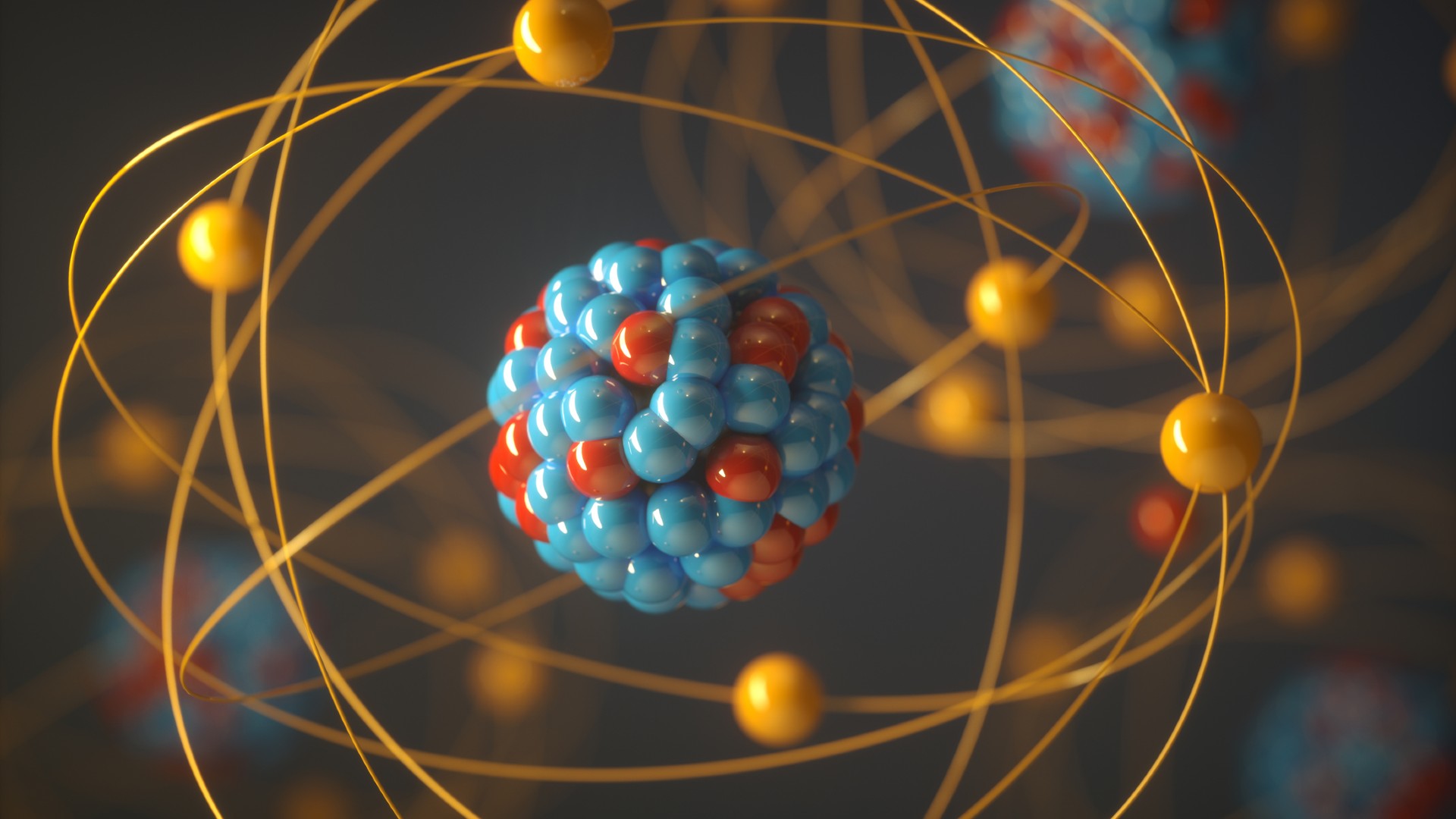
The Standard Model of physics is the theory of particles, fields and the fundamental forces that govern them.
It tells us about how families of elementary particles group together to form larger composite particles, and how one particle can interact with another, and how particles respond to the fundamental forces of nature. It has made successful predictions such as the existence of the Higgs boson, and acts as the cornerstone for theoretical physics.
One way to think about the Standard Model is as a family tree for particles. For example, the Standard Model tells us how the atoms that make up our bodies are made of protons and neutrons, which in turn are made of elementary particles called quarks.
Related: What are bosons?
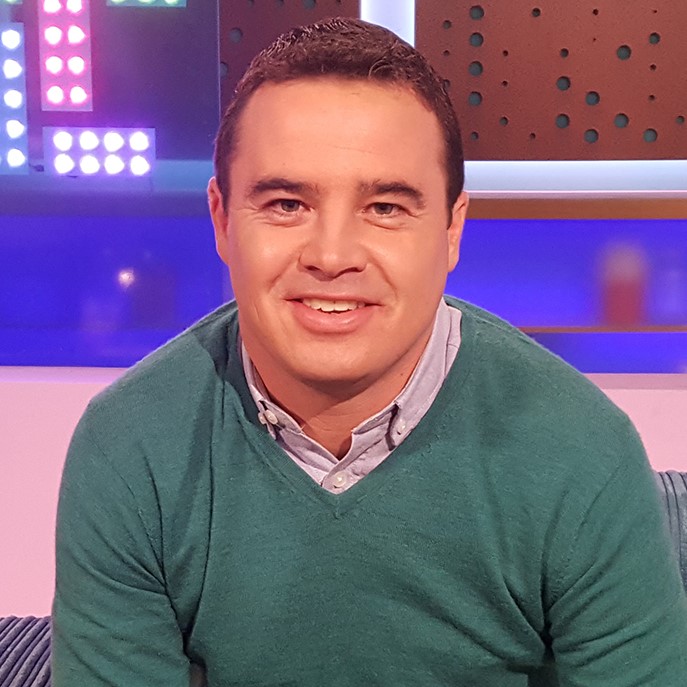
Keith Cooper is a freelance science journalist and editor in the United Kingdom, and has a degree in physics and astrophysics from the University of Manchester. He's the author of "The Contact Paradox: Challenging Our Assumptions in the Search for Extraterrestrial Intelligence" (Bloomsbury Sigma, 2020) and has written articles on astronomy, space, physics and astrobiology for a multitude of magazines and websites.
The Standard Model is considered by physicists, such as Glenn Starkman at Case Western Reserve University, as one of the most successful scientific theories of all time, but on the flip-side, scientists have also recognized that it is incomplete, in the same way that Isaac Newton's theory of universal gravitation derived from his laws of motion, while remarkably successful, was not the whole picture and required Albert Einstein's General Theory of Relativity to fill in the missing gaps.
The History of the Standard Model
The Standard Model was drawn together in the 1960s and early 1970s from the work of a cadre of pioneering scientists, but in truth its origins extend back almost 100 years earlier. By the 1880s, it was becoming apparent that there were positively and negatively charged particles produced when gasses are ionized, and that these particles must be smaller than atoms, which were the smallest known structures at the time. The first subatomic particle to be identified, in cathode rays, was the negative electron in 1897 by the British physicist and subsequent Nobel Prize winner, J. J. Thomson.
Then, in 1911, Hans Geiger and Ernest Madsen, under the supervision of the Nobel Laureate Ernest Rutherford at the University of Manchester, performed their famous 'gold foil' experiment, in which alpha particles (helium nuclei) were fired at a thin gold foil. Some of the alpha particles passed right through the atoms in the foil, while others were scattered left and right and a small fraction bounced right back.
Rutherford interpreted this as meaning that atoms contained a lot of empty space that the alpha particles were passing through, but that their positive charge was concentrated in a nucleus at their center, and on the occasions, an alpha particle hit this nucleus dead on, it was scattered. Further experimentation by Rutherford in 1919–20 found that an alpha particle fired into air could knock a positively charged particle out of a nitrogen atom in the air, turning it into carbon in the process. That particle was the proton, which gives the atomic nucleus its positive charge. The proton's neutrally charged partner, the neutron, was identified in 1932 by James Chadwick at Cambridge, who also won the Nobel Prize.
So, the picture of particle physics in the early 1930s seemed relatively straightforward — atoms were made of two kinds of 'nucleons', in the guise of protons and neutrons, and electrons orbited them.
But things were already quickly starting to become more complicated. The existence of the photon was already known, so technically that was a fourth particle. In 1932 the American physicist Carl Anderson discovered the positron, which is the antimatter equivalent of an electron. The muon was identified in 1936 by Anderson and Seth Neddermeyer, and then the pion was discovered in 1947 by Cecil Powell. By the 1960s, with the advent of fledgling particle accelerators, hundreds of particles were being discovered, and the scientific picture was becoming very complicated indeed. Scientists needed a way of organizing and streamlining it all, and their answer to this was to create the Standard Model, which is the crowning glory of the cumulative work of the physics community of that era.
Particle generations
According to the Standard Model, there are three families of elementary particles. When we say 'elementary', scientists mean particles that cannot be broken down into even smaller particles. These are the smallest particles that together make up every other particle.
The three families are leptons, quarks and bosons. Leptons and quarks are known as Fermions because they have a half-integer spin. Bosons, on the other hand, have a whole-integer spin. What does this mean?
Spin, in the context of quantum physics, refers to spin angular momentum. This is different to orbital angular momentum, which describes Earth's spin around the sun, Earth's spin around its rotational axis, and even the spin of a spinning top. On the other hand, spin angular momentum is a quantum property intrinsic to each particle, even if that particle is stationary. Half-integer spin particles have spin values that are half-integers, so 1/2, 3/2, etc. The bosons have whole integer spin values, eg 1, 2, 3 etc.
Leptons include electrons, muons, tau particles and their associated neutrinos. Quarks are tiny particles that, when joined together, form composite particles such as protons and neutrons. Particles that are made of quarks are called hadrons (hence the Large Hadron Collider), with composite particles formed of odd numbers of quarks, usually three, being called baryons, and those made of two quarks called mesons. Bosons are force carriers — they transfer the electromagnetic force (photons), the weak force (Z and W bosons), the strong nuclear force (gluons), and the Higgs force (Higgs boson).
Each 'family' consists of six known particles (except the bosons, which we'll explain later) that come in pairs called 'generations.' The most stable and least massive particles of the family form the first generation. Because of their stability, meaning that they don't decay quickly, all stable matter in the universe is made from first generation elementary particles. For example, protons are formed of two 'up' quarks and one 'down' quark, which are the two most stable quarks.
There are 17 known elementary particles — 6 leptons, 6 quarks, but only 5 bosons. There's one force carrier missing — the graviton. The Standard Model predicts that gravity should have a force-carrying boson, in the guise of the graviton. Gravitational waves are, in theory, formed from gravitons. However, detecting the graviton will be no mean feat. Gravity is the weakest of the four fundamental forces. You might not think so, after all it keeps your feet on the ground, but when you consider that it takes the entire mass of the planet to generate enough gravity to keep your feet on the ground, you might get a sense that gravity isn't as strong as, say, magnetism can be, which can pick up a paperclip against the gravitational pull of Earth. Consequently, individual gravitons do not interact with matter that easily — they are said to have a low cross section of interaction. Gravitons may have to remain hypothetical for the time being.
Beyond the Standard Model
As wonderful as the Standard Model is, it describes only a small fraction of the universe. The European Space Agency's Planck spacecraft has confirmed that everything that we can see in the cosmos — planets, stars and galaxies — accounts for just 4.9% of all the mass and energy in the universe. The rest is dark matter (26.8%) and dark energy (68.3%), the nature of which are completely unknown and which are definitely not predicted by the Standard Model.
That's not all that's unknown. One big question in physics is whether the elementary particles really are elementary, or whether there is hidden physics underlying them. For example, String Theory posits that elementary particles are made from tiny vibrating strings. Then there's the question of antimatter — equal amounts of matter and antimatter should have been created in the Big Bang, but this would mean we should not be here at all, because all the matter and antimatter should have annihilated each other. Today we see that the universe contains mostly matter, with very little antimatter. Why is there this asymmetry?
Then there's the question of why particles have the masses that they do, and why the forces have the strengths that they have, and why particles are broken down into the three families of leptons, quarks and bosons. That they just are isn't a good enough answer for physicists — they want to understand why, and the Standard Model does not tell them.
Supersymmetry
In an effort to bring the Standard Model up to speed to face these challenges, scientists have introduced the idea of supersymmetry. If true, then supersymmetry would mean that every particle in the Standard Model has a supersymmetric partner with a much greater mass, and a spin that is different by one-half to their Standard Model partners. This would unify fermions with bosons, since the integer-spin fermions would have half-integer-spin super-partners, and the half-integer-spin bosons would have integer-spin super-partners. The least massive and most stable supersymmetry particles would also have no electric charge and interact only very weakly with normal matter, which sounds very much like the properties of dark matter.
Meanwhile, at the very highest energies analogous to those that existed in the first moment after the Big Bang, supersymmetry predicts that the weak force, the strong force and the electromagnetic force would all have the same strength, and essentially be the same force. Scientists call such a concept a 'Grand Unified Theory'.
According to the CERN website, supersymmetry could also help explain the surprisingly small mass of the Higgs boson, which is 125 GeV (125 billion electronvolts). While this is relatively high, it is not as high as expected. The existence of extremely massive supersymmetric partners would balance things out. And they must be extremely massive, because the Large Hadron Collider (LHC), nor any other particle accelerator before it, has found any evidence for the existence of supersymmetric partners so far, leading some scientists to doubt that supersymmetry is real. If supersymmetric particles exist, then they must be more massive than the LHC can detect; for example, the mass of the gluino, which is the supersymmetric partner of the gluon that mediates the strong force binding quarks together inside protons and neutrons, has been ruled out up to 2 trillion eV.
So supersymmetry is in danger and physicists are now scrambling to find a replacement theory that can advance upon the Standard Model and explain the Higgs boson's mass, as well as dark matter, Grand Unified Theories and everything else. There are no strong candidates to replace supersymmetry yet, and supersymmetry may still win out, but for now physicists will have to make do with the imperfect world of the Standard Model.
Additional Reading
Learn more about the standard model with these useful resources from CERN and the Department of Energy. Discover why we should celebrate the detection of a tiny elementary particle with the Institute of Physics.
Follow Keith Cooper on Twitter @21stCenturySETI. Follow us on Twitter @Spacedotcom and on Facebook.
Bibliography
Anderson and Neddermeyer discover the muon. CERN accelerating science. Retrieved September 22, 2022, from https://timeline.web.cern.ch/anderson-and-neddermeyer-discover-muon
Ball, P. (2003, December 5). Centenary of particle pioneer. Nature News. Retrieved September 22, 2022, from https://www.nature.com/articles/news031201-7
Carl D. Anderson. NobelPrize.org. Retrieved September 22, 2022, from https://www.nobelprize.org/prizes/physics/1936/anderson/biographical/
Cathode Ray Tube. Cathode ray tube | University of Oxford Department of Physics. (n.d.). Retrieved September 22, 2022, from https://www2.physics.ox.ac.uk/accelerate/resources/demonstrations/cathode-ray-tube
Ernest Rutherford. NobelPrize.org. Retrieved September 22, 2022, from https://www.nobelprize.org/prizes/chemistry/1908/rutherford/biographical/
J.J. Thomson. NobelPrize.org. Retrieved September 22, 2022, from https://www.nobelprize.org/prizes/physics/1906/thomson/facts/
James Chadwick. NobelPrize.org. Retrieved September 22, 2022, from https://www.nobelprize.org/prizes/physics/1935/chadwick/biographical/
The matter-antimatter asymmetry problem. CERN. Retrieved September 22, 2022, from https://home.cern/science/physics/matter-antimatter-asymmetry-problem
Planck. ESA. Retrieved September 22, 2022, from https://www.esa.int/Science_Exploration/Space_Science/Planck
Planck's new cosmic recipe. ESA Science & Technology. Retrieved September 22, 2022, from https://sci.esa.int/web/planck/-/51557-planck-new-cosmic-recipe
Rutherford, transmutation and the Proton. CERN Courier. (2019, May 8). Retrieved September 22, 2022, from https://cerncourier.com/a/rutherford-transmutation-and-the-proton/
Starkman Glenn Starkman is a Friend of The Conversation. Distinguished University Professor of Physics, G. (2018, May 23). The standard model of particle physics: The absolutely amazing theory of almost everything. The Conversation. Retrieved September 22, 2022, from https://theconversation.com/the-standard-model-of-particle-physics-the-absolutely-amazing-theory-of-almost-everything-94700
Supersymmetric glue: the search for gluinos. CERN Accelerating science. Retrieved September 22, 2022, from https://cms.cern/news/supersymmetric-glue-search-gluinos
Supersymmetry. CERN. Retrieved September 22, 2022, from https://home.cern/science/physics/supersymmetry
Wikimedia Foundation. (2022, August 20). Cross section (physics). Wikipedia. Retrieved September 22, 2022, from https://en.wikipedia.org/wiki/Cross_section_(physics)
Join our Space Forums to keep talking space on the latest missions, night sky and more! And if you have a news tip, correction or comment, let us know at: community@space.com.
Breaking space news, the latest updates on rocket launches, skywatching events and more!
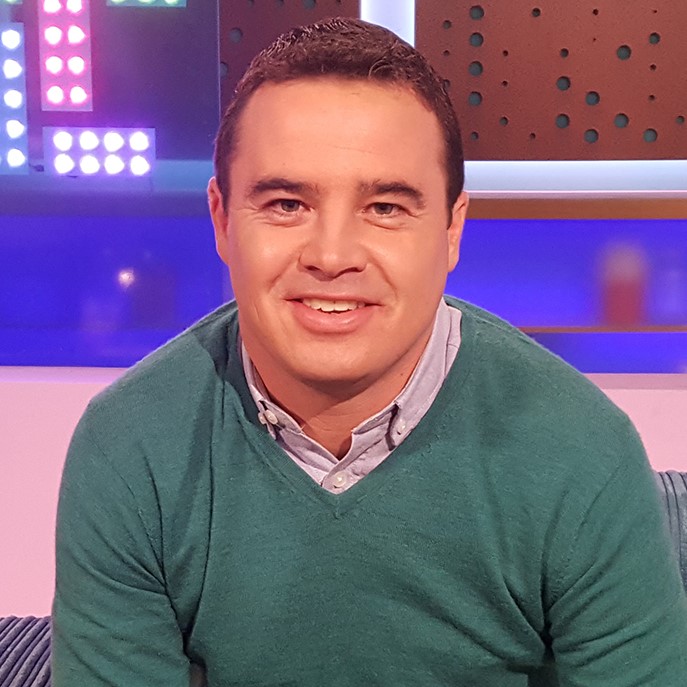
Keith Cooper is a freelance science journalist and editor in the United Kingdom, and has a degree in physics and astrophysics from the University of Manchester. He's the author of "The Contact Paradox: Challenging Our Assumptions in the Search for Extraterrestrial Intelligence" (Bloomsbury Sigma, 2020) and has written articles on astronomy, space, physics and astrobiology for a multitude of magazines and websites.