What are bosons?
Bosons are 'sociable' particle and the cosmos would be a dull place without them
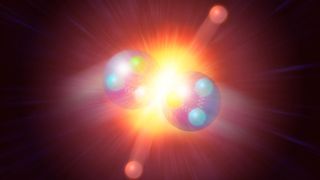
Bosons are particles that carry energy and forces throughout the universe.
The standard model of particle physics — the most robust theory we have of the sub-atomic world — divides every particle in the universe and even the larger composite particles fit into two broad categories; fermions and bosons.
Fermions such as quarks, electrons, neutrinos, protons, and neutrons are the foundation of matter, while one category of bosons, the gauge bosons, are responsible for acting as the 'carriers' of at least three of the four fundamental forces — electromagnetism, the strong nuclear force, and the weak nuclear force. That means that fermions interact with each other via the exchange of gauge bosons.
There may also be a boson to carry the force of gravity, but that isn't currently certain. The gauge bosons are fundamental particles — meaning they're not comprised of smaller particles — but there are other bosons that are composed of smaller particles.
Related: Higgs boson: The 'God Particle' explained
Robert Lea holds a bachelor of science degree in physics and astronomy from the U.K.'s Open University. Robert has contributed to Space.com for over a decade, and his work has appeared in Physics World, New Scientist, Astronomy Magazine, All About Space and more.
Bosons: What makes a particle a boson?
Bosons take their name from Indian physicist Satyendra Nath Bose who conducted important research in the 1920s regarding the behavior of the most famous boson — the photon.
One of the key defining characteristics of bosons relates to a quantum mechanical quality called 'spin' which can be thought of as the deflection a particle takes as it experiences a magnetic field imparting angular momentum.
Though similar, spin is more complex than angular momentum in the macroscopic world of classical physics, mainly because particles can have fractions of spin, meaning there is no real 'classical' way of describing spin.
A fermion is a particle with 1/2 spin that can have plus or minus values. This means that fermions can have values such as 1/2, -1/2, 3/2, and -3/2. The plus or minus determines the direction of intrinsic angular momentum particle will take.
Bosons, on the other hand, have whole integer spins including zero. This means the spin values these particles can take are 0, 1, -1, 2, -2, and so on.
Mathematically adding two halves together makes a whole integer, and in a similar way, combining even numbers of fermions creates a larger particle that is a boson.
These include mesons — which form when two quarks bond — and even atoms with even numbers of fermions. For example, helium-4 atoms are bosons because they consist of two protons, two neutrons, and two electrons. Helium-4 atoms will take a special relevance when thinking about the special and unique properties of bosons.
What are the different bosons?
Bosons can be divided up a few ways, but to introduce the different particles that make up this wing of the 'particle zoo' it's handy to sort them into two rough groupings — particles we have experimental evidence of, and those that are currently just theoretical.
Discovered Bosons
Photons
Easily, the most famous gauge boson is the photon, the constituent particle of light and the mediator of the electromagnetic force.
For photons — which have a spin of 1 — spin is the quantum mechanical equivalent of polarization, or the direction in which a light wave is orientated. This means photon spins can be parallel or anti-parallel in orientation.
Photons were the first gauge bosons to be discovered when at the turn of the 20th century Max Planck and Albert Einstein suggested light exists in packets of energy called 'quanta.' The name 'photon' was introduced for these quanta in 1928 by American chemist Gilbert Lewis.
Related: The double-slit experiment: Is light a wave or a particle?
Gluons
Gluons, the second discovered gauge boson, are the bosons that carry the strong nuclear force. As a result, they are responsible for 'sticking' other particles together.
Specifically, gluons bind quarks together to create protons and neutrons. But gluons don't stop there: They also bind these composite particles — collectively called 'nucleons' — together in the atomic nucleus at the heart of all everyday matter.
Gluons were discovered at the electron-positron collider PETRA of DESY, Germany, in 1979.
The W and Z Bosons
The W and Z bosons are the gauge bosons responsible for carrying the weak nuclear force — stronger than gravity but only effective across incredibly short ranges. These spin 0 bosons are responsible for nuclear decay in which one element changes to another by helping protons change to neutrons and vice versa.
One of the big problems with the W and Z bosons, which were found in 1983, was figuring out how they got their mass, as theories at the time suggested they should be massless like the photon.
Higgs Bosons
The Higgs boson was first introduced into the standard model of particle physics to explain how the W and Z bosons got their mass, but its mass-granting role as the facilitator of the Higgs field was soon extended to almost all particles.
The Higgs boson was discovered in 2012 emerging from high-energy proton-proton collisions at the Large Hadron Collider (LHC) — the world's most powerful particle accelerator.
The Higgs boson has a proposed spin of 0 and its discovery is said to have completed the standard model, but there is still physics outside this model to discover. The exploration of physics beyond the standard model means there are other theoretical bosons to explore.
Theoretical Bosons
Gravitons
One thing the framework of the standard model of particle physics can't describe is gravity. That's because quantum mechanics — the physics of the subatomic — and general relativity, Einstein's theory of gravity, don't mesh.
The other fundamental forces get a gauge boson to carry them (and the weak force even gets two) so why shouldn't gravity? A gauge boson for gravity — the 'graviton' — has been theorized but has thus far failed to manifest experimentally.
Because gravity is negligible at a sub-atomic level, missing gravitons and the lack of a 'quantum theory of gravity' hasn't hindered this model too much.
Boson superpartners
One potential model of physics beyond the standard model is 'supersymmetry.' This theory — proposed to 'fix' the mass of the Higgs boson — suggests that every fermion in the particle zoo has a bosonic partner.
The extra particles would help 'cancel out' some of the mass of the Higgs boson, explaining why it is relatively light.
Bosons: The 'sociable' particles
Thanks to a phenomenon called the Pauli exclusion principle, half-integer spin fermions are incapable of possessing the same quantum numbers. This means that fermions are incapable of bunching together.
Bosons, however, with their full integer spins, don't abide by the Pauli exclusion principle. This means they can closely group together giving rise to some unique physical properties.
The most common example of 'social bosons' is laser light, which is comprised of photons with the same wavelength and frequency all moving in the same direction.
A more exotic example of bosons defying the Pauli exclusion principle was suggested in 1924. Albert Einstein and Bose determined that bosons should condense together in their ground state — the state of their lowest possible energy — leading to Bose-Einstein condensation, the creation of superfluidity in liquid helium cooled to 2.17 K and thus its lowest possible energy.
Coupled electrons — called 'Cooper pairs' — are classed as 'quasi-particles' and can be coerced into behaving like bosons, condensing into a state with zero electrical resistance. The creation of Bose-Einstein condensation in dilute gases of alkali atoms would win three researchers the Nobel Prize in Physics in 2001.
Additional Reading
Explore the Higgs boson in more detail and discover why it's so special with CERN.
Learn more about particle physics with this free course from The Open University.
Bibliography
"Fermions, Bosons." Hyperphysics (2022).
"The Standard Model." CERN (2022).
"Meet a superpartner at the LHC." APS Physics (2010).
"Supersymmetry." CERN (2022).
"Discovery of the Gluon." Sau Lan Wu, University of Wisconsin-Madison/CERN,(2018).
"This Month in Physics History." APS News (2012).
Follow us on Twitter @Spacedotcom or on Facebook.
Join our Space Forums to keep talking space on the latest missions, night sky and more! And if you have a news tip, correction or comment, let us know at: community@space.com.
Get the Space.com Newsletter
Breaking space news, the latest updates on rocket launches, skywatching events and more!
Robert Lea is a science journalist in the U.K. whose articles have been published in Physics World, New Scientist, Astronomy Magazine, All About Space, Newsweek and ZME Science. He also writes about science communication for Elsevier and the European Journal of Physics. Rob holds a bachelor of science degree in physics and astronomy from the U.K.’s Open University. Follow him on Twitter @sciencef1rst.