Atoms: What are they and how do they build the elements?
All the elements in the Periodic Table are made from different atoms, and the structure of these atoms results in a gamut of phenomena from radioactive decay to nuclear power.
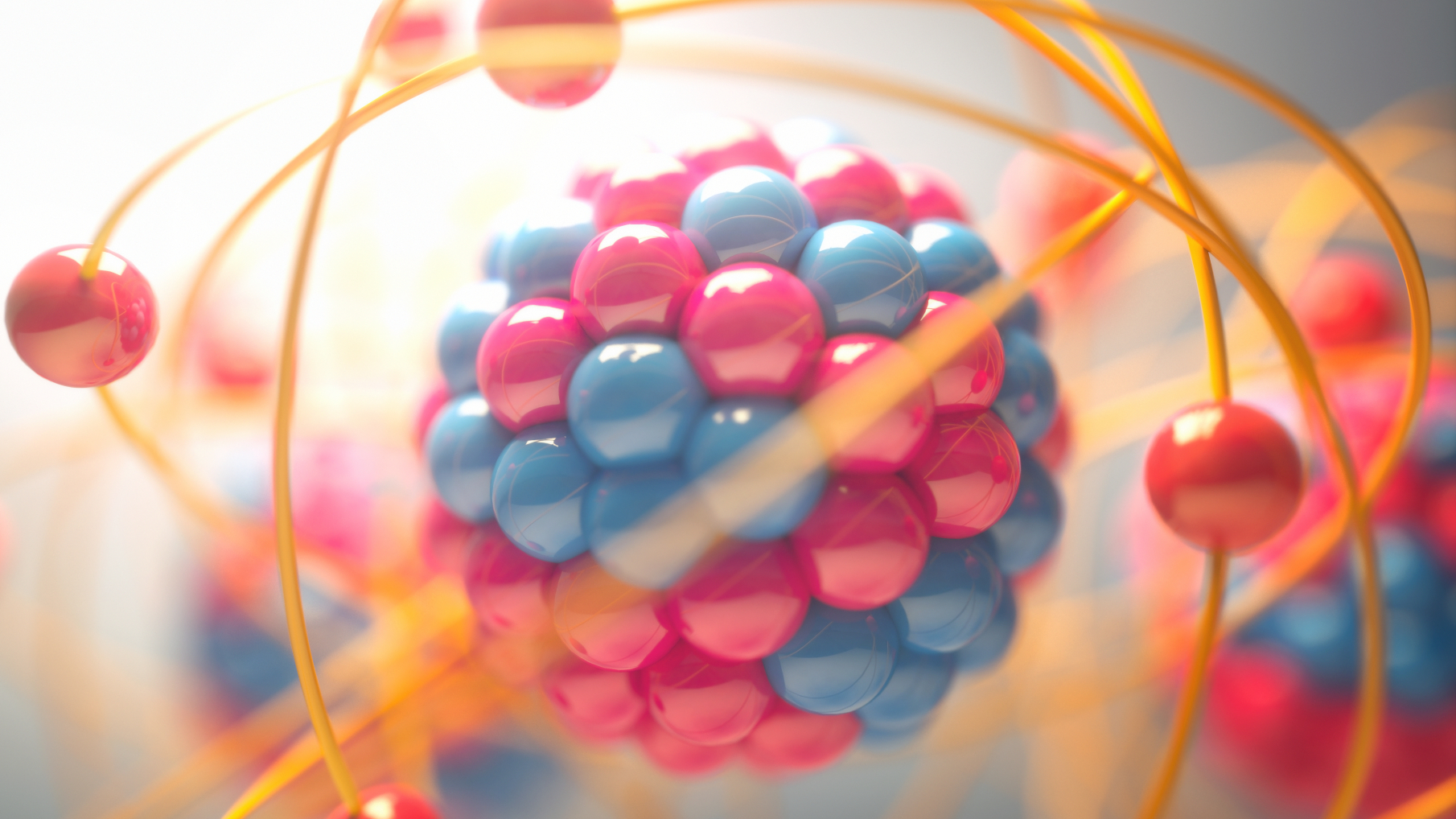
Atoms are the basis of the elements. There are 118 known elements in the Periodic Table, 92 of which occur naturally, and each is based on a different atom.
The word "atom" comes from the Greek "atomos", meaning something that is uncuttable, or which cannot be split. Once upon a time, early scientists and philosophers thought that this was indeed the case, but it turns out that atoms are built from smaller components and can indeed be split, releasing large amounts of energy in the process.
Atoms are also tiny. A typical atom is ten billionths of a meter across. The nucleus of an atom is just a quadrillionth (10^–15) of a meter.
Related: The Large Hadron Collider: Inside CERN's atom smasher
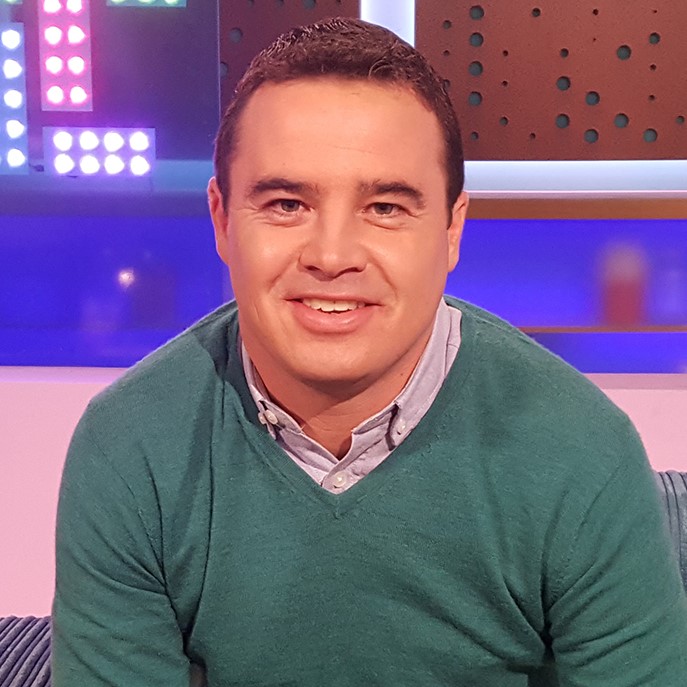
Keith Cooper is a freelance science journalist and editor in the United Kingdom, and has a degree in physics and astrophysics from the University of Manchester.
So how does nature build an atom? The basic picture is of a nucleus containing protons and neutrons surrounded by electrons that orbit around the nucleus. This description is almost correct — because of the uncertainty principle in quantum physics, we cannot actually define an orbit for an electron, and so instead they swarm around the nucleus in an indistinct "cloud". But for simplicity, the basic picture will do for now.
The more protons and neutrons contained in an atomic nucleus, the heavier that element is. So, for example, lead has 82 protons and between 120 and 132 neutrons in its nucleus, whereas hydrogen, the lightest element, has just one proton and occasionally one or two neutrons.
The number of protons in an atom of any given element is always the same. Physicists call this the atomic number. So, in the above example of lead, its atomic number is 82 and that does not change; if it did, it would be a different element (thallium, atomic number 81, or bismuth, atomic number 83). The number of neutrons in the nucleus, as you may have gathered from the above example, can however change, and we call atoms of the same element with different numbers of neutrons "isotopes". Sometimes these isotopes are stable, but often they are not and eventually decay.
Discovery of the atom
It has been known that elements are broken down into basic units of a given weight since the early 1800s, in an insight that came from the English scientist John Dalton. He considered these units to be fundamental, indivisible particles, hence his use of the Greek word "atomos".
Things got more interesting in 1897, when J. J. Thomson discovered electrons, by realizing that cathode rays were streams of particles and not electromagnetic waves. Thomson judged that the electrons must be coming from the atoms within the cathode-ray tube that he was experimenting with. This discovery was of immense importance because it meant that atoms are not the most fundamental particles of all and that they can be formed of smaller particles.
However, atoms are electrically neutral, whereas electrons are negatively charged. So Thomson, reasonably, thought that there must be something inside the atom with a positive charge that was canceling out the negative charges of the electrons. What he envisaged was called the "Plum Pudding Model" with the electrons embedded like plums in the pudding of positive charge.
Things became more defined by 1913. Experiments by Ernest Rutherford, along with his colleagues Hans Geiger of Geiger-counter fame and Ernest Marsden, revealed the truth about atoms. The trio, who were at the University of Manchester, fired what at the time were called alpha particles at a sheet of gold foil. If atoms were structured according to the plum pudding model, where it just contained a mixture of positive and negative charges, the alpha particles should just fly straight through. Instead, Rutherford, Geiger and Marsden found that the alpha particles kept getting deflected, sometimes by a small angle, but sometimes scattered by a large angle, and one in every thousand or so alpha particles bounced straight back.
It should have been impossible for an alpha particle to bounce straight back in the plum pudding model. Instead, Rutherford, Geiger and Marsden interpreted their results as meaning that there was a nucleus of positive charge concentrated at the center of the atom, off which the alpha particles were bouncing.
Based on this, the Danish physicist Niels Bohr, alongside Rutherford, developed an overall model of the atom that depicted electrons orbiting the nucleus in shells corresponding to their energy, and that the remainder of the atom would be empty space. It is the various forces that act across the atom — the strong nuclear force binding the neutrons and protons, and the electrostatic forces of the charged particles, that give the impression of atoms being solid.
In addition, we now know that alpha particles are the nuclei of helium-4, containing two protons and two neutrons, and as we shall see later, they can be the product of radioactive decay.
Electrons in the atom
As we have seen, the number of electrons spinning around a nucleus is usually the same as the atomic number, so that their charges cancel out resulting in a neutral atom (neutrons are also electrically neutral, hence the name, so they don't contribute anything to the overall charge of the atom).
However we picture how electrons move around the atomic nucleus, whether in clean orbits or as a "cloud", we do know that they orbit in shells of increasingly higher energy, and each shell can incorporate up to a certain number of electrons.
The lowest energy shell, which is closest to the nucleus, is called the K-shell and can only fit two electrons. Once the K-shell is filled, any subsequent electrons go first into the L-shell, which can hold 8 electrons, then the M-shell which can hold 18 electrons, then the N-shell and O-shells that can fit 32 electrons each. (Actually, the O-shell could theoretically fit 50 electrons, but there is no known element with this many electrons.)
How atoms become ionized
Electrons are not always confined to their specific shells. If an atom absorbs a photon of light with enough energy — say an ultraviolet photon from a hot star — this absorbed energy can cause an electron to transition to a higher energy level for a short time. This is an unstable situation, however, so the electron drops back down and as it does so, it releases the absorbed energy at a wavelength characteristic of that particular atom.
Sometimes, the energy absorbed by the atom when a photon hits it is enough to not just cause an electron to jump an energy level, but to allow the electron to break free of the atom. The net result is that the previously neutral atom gains an electric charge because the number of protons now exceeds the number of electrons by one (sometimes two, if two electrons are knocked out). This process is called ionization, and an ionized atom is called an ion.
Electron energy transitions and ionization are the processes by which so-called emission nebulae in space glow. Emission nebulae are often star-forming regions, and are being ionized by the hard ultraviolet light from the young, massive stars born within them.
Radioactive decay
Not all atoms are stable. Some isotopes are unstable and will spontaneously decay. There are several types of decay. One is alpha particle decay, wherein an atom releases a helium nucleus. Beta decay involves the emission of a proton or an electron. In gamma-decay, a gamma-ray photon is emitted.
These decay events are random but can be averaged using a term called "half-life". This describes the time it takes for half the amount of any given radioactive material to decay, on average. For example, the half-life of plutonium-238 is 87.7 years, whereas uranium-238 has a half-life of 4.5 billion years.
Splitting the atom
In hindsight, the name "atom", referring to something fundamentally un-splittable, is rather inaccurate. Not only are atoms formed from smaller particles (protons and neutrons, which in turn are formed from quarks, and electrons), but they can also very much be split. Indeed, "splitting the atom" is a common phrase referring to nuclear fission power, and scarily the atomic bomb.
There's a lot of energy wrapped up in an atom. Bombard an atom with enough high-energy particles and it will eventually smash, splitting into two daughter nuclei of lower atomic number and releasing photons of energy in the process. The daughter nuclei produced are different for every fission event. Nuclear reactors bombard atoms of uranium-235 or plutonium-239 with neutrons, and as the atoms split, they produce energy and more neutrons, which can then split other atoms of uranium and plutonium in a chain reaction. Uranium-238 is also fissionable, but "fast" neutrons at higher energies are required to split it. This is often the process used in nuclear weapons.
Nuclear fission results in radioactive nuclear waste in the form of the daughter isotopes produced by the splitting of the uranium or plutonium, which often decay with half-lives of very long timescales, and therefore disposing of this waste is an environmental problem that has not yet been sufficiently solved.
An alternative to nuclear fission is nuclear fusion. As the name suggests, this involves fusing two atoms together to create a heavier atom, releasing energy in the process. There are several advantages of this, including the production of more energy, the non-reliance on radioactive elements such as plutonium that need careful handling, and the fact that fusion produces no radioactive waste. Instead, light elements can be used, such as deuterium (an isotope of hydrogen that has a proton and a neutron) and tritium (an isotope of hydrogen containing one proton and two neutrons), and are in fact preferred. This is because in order to fuse two atoms together, the Coulomb force must be overcome.
The Coulomb force is the electrostatic force felt by like charges — the positive charges of the protons in one atomic nucleus will repel the positive charges of the protons in the other atomic nucleus when attempting to fuse them together. However, with enough energy, this can be overcome, and because nuclei with a smaller atomic number have fewer protons, the Coulomb force is overall weaker and requires less energy to break past. Generating this energy involves creating high temperatures and pressures. Thermonuclear fusion creates a hot plasma — a state of matter formed of ions and electrons rather than the electrically neutral atoms that are found in gas — that can be confined, for example, by magnetic fields in a device called a tokamak, and the pressure in the plasma becomes so great that the nuclei begin to fuse. Another method is inertial confinement fusion, wherein pellets of deuterium and tritium are heated and compressed, often by very powerful laser beams.
Although physicists have achieved controlled nuclear fusion, current experiments are still having to put more energy into the fusion reactions than the amount of energy the reactions are producing. However, there is hope that further experiments, such as at ITER, the International Thermonuclear Experiment Reactor near Marseille in France, will improve techniques to make generating energy through fusion on a large scale possible by the second half of this century.
Sadly, uncontrolled thermonuclear reactions are, however, easier to generate. These are utilized in thermonuclear weapons. More happily, uncontrolled fusion reactions are also what keep the sun and all the stars shining — in the core of a star the pressure of gravitational contraction increases the temperature and pressure high enough to fuse elements all the way from hydrogen up to iron, in a process known as stellar nucleosynthesis. Supernova explosions are even more energetic and can fuse elements heavier than iron.
Additional resources
Read about different types of radiation in more detail with these resources from the Centers for Disease Control and Prevention. A detailed history of alpha particles and Rutherford's work on the atom can be found on the American Institutes of Physics website. Explore atoms in even more detail with The Institute of Physics. If you want to learn more about stellar nucleosynthesis, Oxford University has more information on its philosophy of cosmology site.
Follow Keith Cooper on Twitter @21stCenturySETI. Follow us on Twitter @Spacedotcom and on Facebook.
Bibliography
Particle Physics, by Brian R. Martin (2011, One-World Publications)
The Elements: A Visual Exploration of Every Known Atom in the Universe, by Theodore Gray (2009, Black Dog and Leventhal Publishers)
The Cambridge Encyclopedia Stars, by James B. Kaler (2006, Cambridge University Press)
Join our Space Forums to keep talking space on the latest missions, night sky and more! And if you have a news tip, correction or comment, let us know at: community@space.com.
Breaking space news, the latest updates on rocket launches, skywatching events and more!
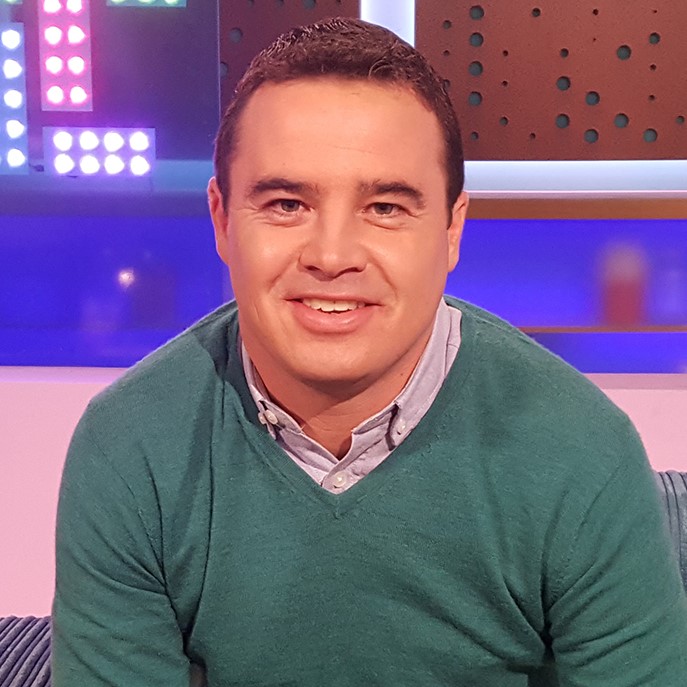
Keith Cooper is a freelance science journalist and editor in the United Kingdom, and has a degree in physics and astrophysics from the University of Manchester. He's the author of "The Contact Paradox: Challenging Our Assumptions in the Search for Extraterrestrial Intelligence" (Bloomsbury Sigma, 2020) and has written articles on astronomy, space, physics and astrobiology for a multitude of magazines and websites.