What is nuclear fusion?
Nuclear fusion supplies the stars with their energy, allowing them to generate light.
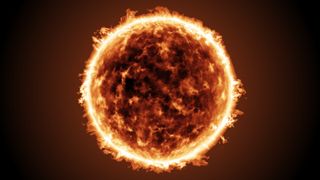
Nuclear fusion is the process of forcing together two light atomic nuclei and creating a heavier one, in the process taking a tiny amount of matter and turning it into massive amounts of energy.
It is nuclear fusion that supplies the stars — including the sun — with their energy, allowing them to generate light. The vast majority of energy that Earth receives comes from the sun, and without it, life itself on our planet would be impossible.
This energy is directed at our planet from what can loosely be described as our star's surface, the photosphere. This layer of the ball of superheated plasma we call the sun is heated by the star's core, where the majority of nuclear fusion takes place. This source of energy is so ubiquitous and so vital here on Earth, that it's little wonder that physicists are desperate to emulate it in reactors on our planet. A future powered by fusion could mean humanity's growing power needs are met by clean and highly efficient fusion energy.
Related: What is the sun made of?
Robert Lea holds a bachelor of science degree in physics and astronomy from the U.K.'s Open University. Robert has contributed to Space.com for over a decade, and his work has appeared in Physics World, New Scientist, Astronomy Magazine, All About Space and more.
Nuclear fusion powers the stars
The key to understanding how fusion generates energy is Albert Einstein's infamous equation explaining how energy equals mass times the speed of light squared (E=mc²). This tells us that matter and energy are interchangeable, while the term c² tells us that a little mass creates a lot of energy.
When matter particles fuse, the particles going into the process have slightly more mass than the daughter particles that are created, with the difference in mass 'liberated' as energy.
Even with the considerable mass-to-energy yield of fusion, each incidence of fusion releases only a tiny amount of energy. Fortunately, stars offset that by having a lot of raw material to power fusion, and these processes run at incredible rates.
The main fusion process that provides the vast majority of the sun's energy is the proton-proton I (PPI) chain. There are two other branches of the PP chain (II and III) but these only account for around 15 percent of the thermonuclear fusion in the sun.
The PPI chain process involves four hydrogen atoms smashing together and creating a helium atom, two electrons, two neutrinos, and two highly energetic gamma-ray photons.
While some of the energy is carried away as the kinetic energy of the daughter particle, the majority is carried by the two gamma-ray photons. These photons will struggle to escape the star's dense interior, however — taking over 30,000 years to move from the core to the surface. During this time the photons are undergoing a series of collisions, absorptions, and re-emissions, which 'downgrade' their energy to photons of visible light eventually radiated out by the photosphere.
Each occurrence of the PPI radiates about 0.0000000000044 Joules, which means — ignoring the other fusion process going on in the sun — our star has to complete this process about 9x10³⁷ (9 followed by 37 zeroes) times every second to maintain its luminosity!
If four grams of hydrogen were converted to helium through this process, only 0.0028 grams would escape as energy. That equates to about 260 billion Joules, enough energy to power a 60-watt light bulb for about 100 years.
Because of its tremendous hydrogen content, the sun has maintained this fusion rate for around four and a half billion years and will continue to do so for a further four and a half billion years until the hydrogen in its center is exhausted.
Related: When will the sun die?
This hydrogen-burning helium forging phase is what astrophysicists call the main sequence lifetime of a star. But, helium isn't the only chemical element being forged in the sun. When and where do stars forge heavier elements?
How does nuclear fusion forge the chemical elements?
Astronomers describe stars as containing hydrogen, helium and everything else (with elements heavier than helium described as 'metals' by astronomers) and these other elements also play a role in fusion.
The PPI isn't the main fusion reaction in more massive stars than the sun, however. Instead, most of these stars' energy comes from the carbon-nitrogen-oxygen (CNO) cycle which requires the higher temperatures of more massive stars to get started.
The CN cycle begins with the nucleus of a carbon-12 atom using it as a catalyst — an element that speeds up a reaction but is unchanged at the end of it — for fusion. Carbon-12 through proton capture goes through various stages until a helium atom is emitted and carbon-12 is recovered. The NO cycle is similar but uses nitrogen-14 as a catalyst.
The energy generated by fusion serves a vital purpose within stars, providing the outward pressure that balances the ball of plasma against the inward force of gravity. That means that when fusion ceases, so goes the outward pressure; this results in the collapse of the star and the swelling and loss of its outer layers.
For stars more massive than the sun — which will end its life as a smoldering white dwarf — this gravitational collapse creates enough pressure to trigger the nuclear fusion of helium created by the main sequence lifetime in its core, fusing it to create carbon, neon and oxygen.
When helium is exhausted, collapse occurs again triggering the fusion of even heavier elements. As this continues, the star develops an onion-like structure with lighter elements fusing in its outer layers and subsequently heavier elements being created towards the core.
This progression of nuclear fusions ends even for the most massive stars when iron dominates the stellar core. This is because iron is an extremely stable element and stars aren't massive enough to trigger its fusion.
When all nuclear fusion ceases, the star undergoes a final and catastrophic gravitational collapse. This triggers a supernova that flings the elements the star has forged during its lifetime out into the universe.
This material from these dead stars becomes the building blocks of the next generation of stars, the planets, and everything around us, including our own human bodies.
Additionally, shockwaves from the compressing iron core — which will eventually birth a neutron star or even a black hole — hit gas shed by the supernova triggering further nuclear fusion creating elements heavier than iron and radioactive materials as well as blasting out x-rays and gamma-rays.
Bringing nuclear fusion power down to Earth
Humanity can't bring the cores of stars down to Earth, so the next best thing is replicating the dense gas of plasma found at the heart of the sun.
The devices designated with the task of doing this here on Earth — nuclear fusion reactors — are called tokamaks. Tokamaks are often also called 'artificial suns' due to the fact these doughnut-shaped machines replicate processes that occur in the sun.
There are currently over 200 tokamaks in operations across the globe, with the scientific milestones achieved in these devices developing a roadmap for the operation of the International Thermonuclear Experimental Reactor, or ITER , the world's largest fusion experiment under construction in the south of France.
A commercial tokamak will aim to use the thermal energy of a plasma heated by fusion to heat water, create steam and in turn spin a turbine that generates electricity.
Though fusion can involve a wealth of chemical elements, the nuclear reaction that most tokamaks aim to make viable is the fusion of the heavy hydrogen isotopes deuterium (with a nucleus of one proton and one neutron) and tritium (one proton and two neutrons). Fusing atoms of these elements together creates a neutron and a helium nucleus.
Part of what makes fusion such a promising source of energy is the fact that deuterium is easily extracted from ordinary seawater. The International Atomic Agency (IAEA) estimates that enough deuterium can be extracted from 0.26 gallons (one liter) of water to provide as much energy as the combustion of 79 gallons (300 liters) of oil. That means the oceans contain sufficient deuterium to sustain humanity's fusion energy needs for millions of years.
Tritium, on the other hand, can be made from lithium, also abundant in nature.
In addition to this, the main byproducts of fusion power, neutrons and helium, are not radioactive and thus don't present the same disposal problems as the byproduct of nuclear fission plants — with fission being almost the mirror image of fusion, breaking large atoms apart into smaller, often radioactive atoms.
Fusion by-products also don't have a significant environmental impact, unlike the greenhouse gases created by the burning of fossil fuels — a major contributing factor in human-driven climate change.
The question is; if fusion power is so good, why don't we already have it?
Why don't we already have nuclear fusion reactors?
Fusion processes aren't easy to replicate here on Earth partially because massive forces of gravity within stars are needed to overcome the repulsion between positively charged atomic nuclei of hydrogen.
That incredible gravitational pressure can't be reproduced here on Earth, so instead the designers of tokamaks must generate fusion in plasmas at incredibly hot temperatures, vastly greater than those at the heart of the sun, to drive nuclei close enough to fuse.
The target temperature for plasmas at tokamaks is around 270 million degrees Fahrenheit (about 150 million degrees Celsius). That's about 100 times the temperature at the core of the sun, about 27 million degrees Fahrenheit (15 million degrees Celsius).
The current temperature record for tokamak is held by China's EAST tokamak which in late 2021 was able to generate plasma at a temperature of about 216 million degrees Fahrenheit (120 million degrees Celsius) for 101 seconds. During this time the plasma briefly reached a peak temperature of about 288 million degrees Fahrenheit (160 million degrees Celsius).
Extreme temperatures aren't the only thing tokamaks have to generate to replicate the gravitational influence of the sun, however. Superheated plasma has to be contained, and to do this tokamaks use incredibly powerful magnetic fields. Currently, it takes more energy to generate these fields than scientists can get out of fusion.
The record for fusion energy generation here on Earth was set by the Joint European Torus (JET) lab in Oxfordshire, England in Febuary 2022. The tokamak was able to generate 59 megajoules of energy using a deuterium-tritium fuel mix in an experiment that lasted just over five seconds.
Any tokamak looking to meet actual energy demands will have to sustain superheated plasma for much longer periods than this, with the main aim being to create a self-sustaining plasma.
If all goes according to plan, ITER will be the first fusion reactor to produce net energy, which means producing more energy than it takes to generate superheated plasma and keep it contained in a powerful magnetic field.
Additional Resources
What are tokamaks, the devices that contain superheated plasma to generate fusion? Read more at the U.S. Department of Energy's website.
Bibliography
"What is Nuclear Fusion?" IAEA (2022).
"DOE Explains...Nuclear Fusion Reactions." U.S. Department of Energy Office of Science (2022).
"An Introduction to the Sun and Stars." Jones. M.H. & Green. S. F. (2003). Cambridge University Press.
"Stellar Evolution and Nucleosynthesis." Ryan. S. G. & Norton. A.J. (2010). Cambridge University Press.
"60 Years of Progress." ITER (2022).
"The Sun and Nuclear Fusion." Western Washington University (2022).
"Old Stars." ASPIRE (2022).
"Fusion energy record demonstrates powerplant future." GOV.UK (2022).
Follow us on Twitter @Spacedotcom or on Facebook.
Join our Space Forums to keep talking space on the latest missions, night sky and more! And if you have a news tip, correction or comment, let us know at: community@space.com.
Get the Space.com Newsletter
Breaking space news, the latest updates on rocket launches, skywatching events and more!
Robert Lea is a science journalist in the U.K. whose articles have been published in Physics World, New Scientist, Astronomy Magazine, All About Space, Newsweek and ZME Science. He also writes about science communication for Elsevier and the European Journal of Physics. Rob holds a bachelor of science degree in physics and astronomy from the U.K.’s Open University. Follow him on Twitter @sciencef1rst.