How Water Made Earth Livable for Us - The Peroxy Way
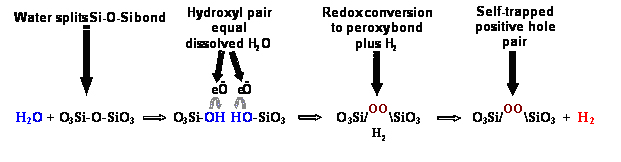
Living on a planet with an oxygen-richatmosphere we tend to forget that our planet is an anomaly.
About 4.5 billion years ago, when the solarsystem accreted out of a disk of gas and dust, the Earth was thoroughlyreduced. Over the course of the first 1-2 billion years our planet becameslowly, but inextricably ever more oxidized. Vast amounts of iron richsediments precipitated out of the oceans, known as ?Banded Iron Formations? orBIFs, indicating that reduced ferrous iron, Fe2+, converted intoferric iron, Fe3+. This required a large, sustained supply ofoxidizing power.
In his 1984 book, ?The Chemical Evolution of the Atmosphereand Oceans,? H. D. Holland estimated that, over the 2+ billion years during which theBIFs precipitated, at least 1012 gram oxygen had to be injected intothe Earth?s oceans every year. Sometime around 2.4 to 2.3 billion years ago,the global oxidation accelerated. During this remarkable period, known as the?Great Oxidation Event,? free O2 appeared in Earth?s atmosphere andsoon increased to the over 20% O2, which we now enjoy.?
The Great Oxidation Event is attributed to theinvention of photo?synthesis: the capacity of living organisms, using sunlight,to split H2O and CO2 into O2 plus reduced Hand C, which in turn combine to produce organic compounds. New forms of lifeappeared that could harness the newly available chemical energy: firstmicrobial and later multi-cellular organisms prospered in the oceans andeventually conquered the land.?
If the Great Oxidation Event can be linked tooxygenic photo?synthesis, the question remains what process might have driventhe earlier slow oxidation of Earth.
One school of thought has promoted the idea thatsome form of oxygenic photo?synthesis was invented very early on, soon afterthe origin of life. Maybe colonies of photosynthetic bacteria, similar totoday?s cyanobacteria, were building stromatolites in shallow waters along thecoasts of early continents, pumping out enough oxygen to precipitate the BIFsand prepare the way for the stupendous rise of free O2 in Earth?satmosphere during the Great Oxidation Event.
The ?invention? of oxygenic photosynthesis soearly in Earth?s history poses serious problems. Oxygen is one of the mostreactive elements in nature, and is toxic to life adapted to reducingenvironments. Before pumping out oxygen as part of their metabolism, themicroorganisms must have learned how to handle this dangerous by-product oftheir cellular biochemistry and how to extract the energy. They must havelearned how to detoxify those Reactive Oxygen Species, commonly referred to inmicrobiologists? circles as ROS, which are the scourge of all forms of life.?
Get the Space.com Newsletter
Breaking space news, the latest updates on rocket launches, skywatching events and more!
One possible solution to this dilemma is that,long before the Great Oxidation Event, the Earth might have been slowlyoxidized by some non-biological process. Such a process would have given themicroorganisms time to adapt to the changing environment or, as Dr. LynnRothschild of the NASA Ames Research Center said, ?It would have provided atraining ground for early life to learn how to handle oxygen.?
Indeed, such a non-biological process exists.When rocks crystallize from magmas that contain dissolved gases, mostly H2O,or when minerals re-crystallize at high temperatures in H?2O?ladenenvironments, water becomes an impurity in their crystal matrices, usually inthe form of hydroxyl, OH?. Even minerals that do not normally containhydroxyl invariably take up small amounts of water giving O3Si-OH,more generally O3X-OH, where X can be Si4+, Al3+,etc.? Most of those will occur in the form of O3X-OH OH-XO3pairs.?
In the Earth Sciences redox reactions arebroadly discussed, usually involving transition metal cations that change theirvalence states such as Fe2+ oxidizing to Fe3+.? Redoxreactions involving anions are also quite popular such as reactions with sulfurthat can change from sulfide, S2-, to sulfate, SO42-,where sulfur is in the valence state S6+.? However, for some unknownreason, oxygen anions are always considered to be frozen into their 2? valencestate, O2-.
Years ago, while studying impurity hydroxyls inMgO, I discovered an unusual redox reaction that involves OH? pairs:during cooling OH? pairs in the MgO matrix change into peroxyanions, O22?, plus H2, as indicated in Equation1.? In other words, two oxygens change their valence from 2- to 1-, meaningthat they become oxidized, while two protons become reduced to molecular H2:
OH? + OH???? O22-+ H2
[Equation 1]
Insubsequent years it became clear that hydroxyls in silicate minerals, also dueto some ?water? being incorporated during crystallization orre-crystallization, undergo the same type of redox reaction, oxidizing twooxygens to the peroxy state while splitting off an H2 molecule, as depicted in thisgraphic of Equation 2.
H2is capable of diffusing away over time, even escaping to grain boundaries andbeyond. Thus, an interesting situation arises: Rocks that contain minerals withimpurity hydroxyl ? essentially any rock ? will acquire peroxy as a memory oftheir solute H2O content. A peroxy, however, is nothing but an extraO atom stored in the mineral structure, equivalent to half an oxygen molecule,O2:
O3Si-OO-SiO3??? O3Si-O-SiO3+ ? O2
[Equation 3]
There are numerous consequences. One is rootedin semiconductor physics.? A peroxy is composed of two O?, which aretightly bound together and inactive for all practical purposes. However, when aperoxy bond breaks, the rock becomes a semiconductor. The reason is that an O?in a matrix of O2- is a defect electron or ?hole?. It is associatedwith energy levels in the valence band of the otherwise insulating silicateminerals. All mineral grains in a rock that are in physical contact with othersare also in electric contact, as far as their valence bands are concerned. Inother words, a hole in any given mineral grain in a rock is able to pass to anyneighboring grains. In fact, the holes associated with O? stateshave been shown in the laboratory to travel through meters of rock as well asthrough sand and soil. There is little doubt that these electronic chargecarriers are able to travel large distances through the Earth?s crust, throughtens of kilometers at least.????
All that is needed for these electric currentsto start flowing are: (i) a process to break the peroxy bonds, (ii) a pathwayfor the charge carriers to flow.
It has been shown that stressing rocks causes peroxybonds to break and to release hole charge carriers that travel fast and far. Thisphotograph shows a 4-meter long piece of granite squeezed at one end. Uponrunning a wire from the stressed end to the front end, where a copper electrodeis attached, a current of about 1 nanoampere is obtained. This current runsalong the stress gradient for hours, even days, as long as the load on the rockis kept constant. Taking off the load causes the current to fade. Re-stressingthe rock causes to the current to come back. The process can be repeated manytimes. The rock is a battery that is charged by stress and can be recharged byre-applying stress.
When we replace the copper contact with a waterbath, into which we introduce a copper electrode, the same current flows. Usinga slab of gabbro, a rock mineralogically similar to basalt, we measure acurrent on the order of 100 nanoamperes. It has been flowing for over 4 weekswithout loosing more than 30% of its initial strength. However, with water, wesee a new reaction: the holes that flow through the rock and pass through therock-water interface oxidize water to hydrogen peroxide, H2O to H2O2.The reaction is quantitative, generating one H2O2 moleculefor every two hole charge carriers that cross the rock-water interface.
What does this mean for the early Earth? Thegeological literature provides convincing evidence that our home planet hasbeen tectonically active since the earliest times. There must have beenplenty of tectonic stresses acting on the rocks that built the continents.There must have been plenty of stress gradients, along which the same type ofhole currents were flowing that we can now demonstrate in laboratoryexperiments. Wherever these currents crossed rock-water interfaces, forinstance along continental margins at subduction zones or othermountain-building regions, water must have been oxized to hydrogen peroxide,which in turn decomposes rapidly into water plus oxygen:
H2O2? H2O + ? O2
[Equation 4]
This electrochemical oxidation of water musthave helped our planet to become ever more oxidized, contributing to the earlyslow oxidation of Earth.
Yet, electrochemical oxidation of water wassurely not the only reaction that pushed the early Earth toward an ever higherdegree of oxidation. Global weathering has to be taken into consideration, too.Weathering is a powerful process that dissolves rocks and wears down mountains.Today about 3 km3 of rocks pass through the global weathering cycleevery year. When the Earth was young, the continents were bare and the rain wasmore acid than today due to the higher CO2 content in the earlyatmosphere. Hence, weathering rates must have been higher too, say 10 km3per year. When weathering eats into a rock, water hydrolyzes the peroxy andproduces hydrogen peroxide, even if the H2 molecules formedaccording to equation 5 still linger around:
O3Si-OO-SiO3+ 2 H2O? ?? O3Si-OH + OH-SiO3 + H2O2
[Equation 5]
If we take a conservative estimate for theaverage peroxy content in rocks, 300 parts per million, the amount of H2O2released globally at a weathering rate of 10 km3 per year translatesinto 1013 grams per year. This is 10x the amount that H. D. Hollandestimated to be necessary to precipitate the BIFs.??
Thus we come to the tentative conclusion that,through weathering and electrochemistry, peroxy in rocks provided enoughoxidation power to change the course of our planet?s history. Over the courseof 1.5 to 2 billion years, peroxy forced the early Earth to slowly butinextricably become ever more oxidized.? Along the way dangerous ReactiveOxygen Species, constantly produced at rock-water interfaces and during peroxyhydrolysis, challenged the early microbes, archaea and bacteria. As Dr. Rothschildso aptly put it, the ROS might have provided a ?training ground? for thoseearly micro-organisms to learn how to deal with oxygen. They developed thebasic enzymatic defenses, which our bodies still use today to fend off thedetrimental side effects of our oxygen-based metabolism.
Thus, while the Earth was still overwhelminglyreduced, eukaryotes joined the archaea and bacteria. Under the onslaught ofthose ROS, the eukaryotes ?learned? how to survive in an oxygen-spikedenvironment long before free O2 gas appeared in Earth?s atmosphere.At some point the eukaryotes learned how to take advantage of the largechemical energy that oxygen can provide. They adapted to do oxygenicphotosynthesis, to tap the energy in O2. This lead to the GreatOxidation Event and to plenty of free O2 in Earth?s atmosphere thatmade our planet livable for us?and it all started with water and a little-knownsolid state reaction in the rocks.
- Video - Reflections on Fermi's Paradox
- How I Spent My SETI Summer
- Video - Listening for Life
Join our Space Forums to keep talking space on the latest missions, night sky and more! And if you have a news tip, correction or comment, let us know at: community@space.com.